A Comprehensive Overview of Accelerator Mass Spectrometry
Accelerator mass spectrometry (AMS) was developed in the 1970s for the analysis of 14 C in environmental and archaeological materials, despite its first demonstration occurring in the 1930s. Presently, it is mainly used for a limited number of isotopes, although it has the potential to be modified for a wider variety of analytes. AMS represents the integration of high-energy physics with radioanalytical chemistry and finds applications in areas such as geoscience, environmental research, archaeology, cosmochemistry, biosciences, medicine, materials science, nuclear chemistry, and physics. AMS employs a high-energy tandem accelerator to increase ion energies into the mega-electron-volt (MeV) territory, which allows for total annihilation and eradication of isobaric interferences. Standard AMS is capable of measuring extremely low isotope ratios, generally between 10 -10 to 10-15 (atomic ratio). The isotopes of interest include 14C, 10 Be, 26Al, 36Cl, 41Ca, and 129 I. The naturally occurring ratios of these isotopes are considerably lower than the 1–10 -9 range seen with other mass spectrometry techniques, except for laser-based systems using resonance ionization methods that can reach similar levels to AMS. Accelerators were invented to strike targets with high-energy ions for fundamental physics research. Researchers discovered that the techniques for creating, selecting, and steering ion beams at mega-electron-volt energy levels could be harnessed to measure very small isotope ratios with high sensitivity and low background noise. The AMS technique provides remarkable selectivity and efficiently eliminates interferences by completely removing the interference from molecular species. The initial acceleration of negative ions plays a role in this process, as most molecular ions and several atomic isobars are unstable as negative ions at elevated energies. Conversely, other mass spectrometric techniques can be affected to varying degrees by isobaric interference from ionic molecules that are nearly identical in mass. High mass resolution can be leveraged to differentiate between molecular and isotopic ion species with slight mass differences, though this often comes at the cost of decreased sensitivity and increased complexity of the system. Recent progress in ion–molecule reaction chemistry, such as those showcased by collision/reaction cell ICPMS (inductively coupled plasma mass spectrometry), can also achieve high mass resolution. These techniques may provide isotope ratio measurements that sit between conventional mass spectrometry and AMS. An AMS is made up of an ion source, an input mass or momentum selector (commonly a magnetic sector), a high-energy accelerator, a ‘stripper’ (a low-pressure gas or thin foil situated in the center of the high-voltage area to remove electrons from the incoming ions), post-accelerator ion optics and mass selectors, along with one or more detection systems. The high-energy accelerator is typically a tandem accelerator, which features two high-energy accelerators positioned back-to-back with a stripper in between them. The voltage rises from zero at one end to the center and then drops back to zero at the opposite end. Low-energy negative ions are introduced at one end of the accelerator, stripped of their electrons, and then reaccelerated to emerge as high-energy positive ions at the other end. This tandem acceleration setup allows both the ion source and the ion detection areas to be operated at or close to ground potential, simplifying the construction and electrical functioning of the instrument. Ions are generally created using a secondary ion or sputter source, often employing a Cs ion source (the type commonly used in secondary ion mass spectrometry) to generate negative ions. These ions are extracted, focused, and initially accelerated to energies between 10 and 100 keV. The input mass selector channels a specific sputtered ion mass into the tandem accelerator, which then boosts these negative ions to mega-electron-volt energies. A stripper located in the central section of the accelerator breaks down molecular ions and eliminates multiple electrons from the ions, transforming incoming negative ions (whether elemental or molecular) into outgoing multiply-charged positive ions. These positive ions are further accelerated by the same potential and exit from the back end of the accelerator with energies that can exceed 30 MeV, depending on the base voltage (or potential) of the accelerator and the level of ionization. Charge states of +10 or greater can be generated, particularly for heavy ions such as actinides. Following acceleration, additional ion optics and switching magnets are utilized to select the ion mass and charge state, directing these ions into detectors for measurement. During operation, both the abundant and rare isotopes are measured one after the other by switching peaks from one isotope to another, similar to other mass spectrometers, in order to ascertain atom ratios. The primary isotope can generate a current in the microampere range, while the rare isotope may yield a signal as low as a few counts per minute. The initial isotope ratio is derived from the counted pulses divided by the integrated current. Calibration using measurement standards with established isotope ratios offers the factor necessary to convert the initial ratio into an atom ratio. Distinct characteristics of the AMS include ion energy levels in the mega-electron-volt range, in contrast to the kilo-electron-volt range or lower seen in other mass spectrometers, the transformation of negative ions into positive ions, and the production of multicharged ions. The rare and primary isotopes can be measured in different charge states, influenced by the element being analyzed, the need to mitigate isobaric interference, and the instrument's configuration. Nonetheless, the high energy involved raises safety and health concerns. The ions in an AMS possess enough energy to create radionuclides and radiation through nuclear interactions. Ions that come into contact with various components of the system (such as slits, deflection plates, and the inner surfaces of the vacuum system) can lead to the generation of neutrons and x-rays. High voltages are linked to the ion source, deflection plates, and other sections of the instrument. These aspects necessitate stringent adherence to radiological and electrical safety protocols and protective measures. The AMS method is utilized to quantify rare isotopes in various samples, including air, water, ice, soil, minerals, meteorites, biological tissues, and archaeological items. Typically, the size of the elemental samples falls within the milligram range to ensure sufficient rare isotope count rates for effective analysis. Prior to analysis, samples are generally treated to extract and purify the target element along with its associated rare isotope. In some cases, it may be necessary to add a carrier for processing and yield assessment, such as iodine for 129I analysis in seawater; however, it is crucial to measure the rare isotope background present in the carrier. In contrast, the addition of a carrier is not needed for the analysis of 14C, as the samples contain enough carbon for the analysis and the objective is to ascertain the 14C/ 12C ratio within the sample. The purified element is then transformed into a solid form that is suitable for generating negative ions via Cs sputtering. The rare isotope's element under investigation can be analyzed either in its molecular form or as a reduced sample, depending on its ionization behavior. For example, carbon is examined in the form of graphite, while iodine is evaluated as silver iodide. The resulting solid is compacted into a metal holder, usually made of aluminum or copper, and positioned in the AMS ion source for further analysis. The uncommon isotopes 14C, 10Be, 26Al, 36Cl, 41Ca, and 129I constitute the majority of the tasks carried out by AMS laboratories, with 14C accounting for the overwhelming share of these activities. Recently, AMS has been utilized for the analysis of heavy elements, such as uranium and plutonium. Additional isotopes that AMS analyzes include tritium, 59Ni, 90Sr, and 205Pb. Detection thresholds for AMS are generally expressed as limiting isotope ratios, which represent the measured quantities in AMS. The overall ion transmission of a typical AMS results in absolute atom detection efficiencies that are lower than those of TIMS or ICPMS, roughly around 1 count per 100,000 atoms introduced into the device. However, the remarkably low background of the AMS methodology allows for detection and quantification at the subfemtogram level, comparable to the finest ICPMS and TIMS results. For certain radionuclides, a direct measurement is sufficient, relying on calibration with a radionuclide standard and referencing the mass of the measured sample. In contrast, other radionuclides require the isotope ratio with their stable counterparts. A more intricate scenario involves measuring the 14C/ 12C isotope ratio in an environmental or archaeological sample against the current atmospheric CO2 value. This ratio needs to be corrected for anthropogenic 14C generated from nuclear weapon testing and other nuclear activities, as well as for variations in atmospheric CO2 due to cosmic-ray flux changes over time. For elements like plutonium, which lack stable isotopes, the total and absolute quantity is determined through isotope dilution mass spectrometry, where the sample is traced (or spiked) with 242Pu or 244Pu. Accelerator Mass Spectrometry (AMS) has emerged as a significant analytical technique in drug development, offering several advantages over traditional methods like Liquid Scintillation Counting (LSC). Here are the key applications and benefits of AMS in this field: AMS allows for a significantly lower amount of radioactivity to be administered to human volunteers. This is particularly beneficial when the drug shows retention of radioactive materials in nonclinical studies, enabling safer clinical trials with minimal radiation burden to subjects. The ability to use lower doses of radioactivity permits the administration of multiple doses, whether as pulse doses or repeated doses. This flexibility is crucial for studies requiring various dosing regimens. AMS's high sensitivity enables researchers to monitor pharmacokinetics and excretion over extended periods, allowing sample collection for weeks or even months post-dosing. This contrasts with conventional methods, which typically limit sample collection to about one week. With AMS, subjects can be released from clinical settings once their excretion levels of the radioactive drug reach acceptable limits. This is a significant improvement over traditional methods, where higher doses necessitate longer observation periods. AMS has revolutionized the design of IV microtracer studies, facilitating the administration of drugs via alternative routes, such as ocular and dermal applications. This capability broadens the scope of drug delivery methods that can be explored. AMS is instrumental in assessing absolute bioavailability, which is essential for understanding a drug's pharmacokinetics in systemic circulation. These assessments can influence decisions regarding the progression or termination of drug development based on the drug's absorption and metabolism characteristics. The application of AMS in biomedical research began in the 1990s, marking a shift from research-based applications to its use as a standard analytical technique in drug development. AMS provides a highly sensitive and flexible approach to drug development, significantly enhancing the safety and efficiency of clinical studies while offering critical insights into pharmacokinetics and drug behavior in the body. The evolution of Accelerator Mass Spectrometry (AMS) has been marked by significant advancements in instrumentation and applications, particularly in the fields of research and drug development. Here are the key developments in the evolution of AMS: Over recent decades, AMS instruments have undergone substantial simplification, leading to reduced size and complexity while enhancing sensitivity tailored to specific applications. This evolution has made AMS more accessible for various research fields. AMS instruments have been specifically designed for carbon analysis, allowing for precise measurements of carbon isotopes. Larger AMS systems can analyze a broader range of elemental isotopes due to their higher energies, which has expanded the use of AMS in modern research. Today, high-performance AMS instruments are operational in over 100 facilities worldwide, although their application in biomedical research remains limited to a few specialized centers. Historically, voltages below 2.5 MV were considered inadequate for dissociating molecular background interferences. However, the ETH Zurich group demonstrated that low background carbon AMS could be achieved with 0.5 MV tandem accelerators, leading to the development of the 250 kV single-stage AMS. The first carbon-dedicated AMS system was built around 2003, achieving precision in measuring 14C:12C ratios suitable for biological sample analysis. This prototype paved the way for the commercially viable 250 kV SSAMS. A significant advancement in AMS technology was the direct introduction of gas (carbon dioxide from combusted samples) using a helium carrier. This method eliminated the resource-intensive graphitization process, improving sample throughput and sensitivity, particularly beneficial for metabolite profiling in drug development. While sample-to-sample carryover remains a concern in gas analysis, ongoing research aims to manage and reduce this issue through further instrument development. The technological advancements in AMS are expected to enhance drug development research, especially in pediatric applications. The evolution of AMS has led to significant improvements in instrumentation, sensitivity, and application flexibility, making it a vital tool in modern research, particularly in drug development. Accelerator Mass Spectrometry (AMS) is a powerful technique, but it does have several limitations that researchers should consider: AMS typically requires a sample size of between 0.5 and 2 mg of carbon for reliable measurements. This can be a limitation when dealing with samples that have low carbon content, such as urine, which usually contains less than 0.5% carbon. In such cases, additional carbon must be added, complicating the analysis. The need for sample preparation, including the addition of a carbon carrier for samples with low inherent carbon, can introduce variability and potential errors in the results. This step is crucial for ensuring accurate quantification but adds complexity to the process. Currently, there are only a few AMS systems capable of automated analysis. This limitation can hinder throughput and efficiency in laboratories, especially when high sample volumes are required. Although advancements have been made, background interferences can still pose challenges. For instance, voltages below 2.5 MV were previously thought inadequate for dissociating molecular background interferences, which can affect the accuracy of measurements. The sensitivity of AMS can vary significantly depending on the sample type and its composition. For example, differences in background 14C content between sample types can affect the limit of quantification, making it essential to account for these variations during analysis. While AMS can provide rapid results, the analysis times can still be around 4 minutes per cathode for biomedical work. This may not be suitable for all applications, especially those requiring real-time results. There is a scarcity of publicly available data demonstrating the potential improvements of AMS over traditional methods like graphite. This lack of data can make it challenging for researchers to fully understand the advantages and limitations of AMS. These limitations highlight the need for careful consideration when choosing AMS for specific applications, particularly in drug development and environmental studies. Accelerator Mass Spectrometry is a revolutionary tool in scientific research. Its applications in archaeology, medicine, and environmental science continue to expand, offering unparalleled precision and efficiency. The primary use of Accelerator Mass Spectrometry (AMS) is in drug development, where it provides highly sensitive analysis of pharmacokinetics and excretion profiles of drugs. This technique allows for the administration of very low levels of radioactivity, reducing the radiation burden on human subjects and enabling extended sample collection periods, which enhances the understanding of drug behavior in the body. AMS dating is highly accurate, providing precise measurements of radiocarbon levels in samples. The technique allows for the analysis of very small amounts of material, typically just a few milligrams, which enhances its sensitivity compared to traditional methods like liquid scintillation counting (LSC). This increased sensitivity enables reliable dating of samples, making AMS a valuable tool in fields such as archaeology and environmental science. AMS is superior to traditional radiocarbon dating methods primarily due to its increased sensitivity and ability to analyze much smaller sample sizes, often just a few milligrams. This allows for more precise measurements of carbon isotopes, particularly the 14 C: 12 C ratio, which is crucial for accurate dating. Additionally, AMS significantly reduces background interference, leading to more reliable results, especially in complex samples. These advancements make AMS a preferred choice in various research fields, including archaeology and environmental studies. Costs vary but typically range between $300 and $1,000 per sample. AMS is specifically designed to detect carbon isotopes, particularly 12 C, 13 C, and 14 C. It measures the ratios of these isotopes to ensure stable transmission throughout the analysis, but it does not detect all isotopes universally. The focus on carbon isotopes is crucial for applications like radiocarbon dating and biomedical research, where precise measurements of these specific isotopes are essential for accurate results.1.Introduction to Accelerated Mass Spectrometry
2.AMS Instrumentation
3.AMS Sample Preparation
4.AMS Applications
5.Application of AMS to Drug Development
Reduced Radiation Exposure:
Flexibility in Study Design:
Extended Sample Collection:
Safe Discharge of Subjects:
Support for Concomitant Dosing:
Critical Bioavailability Assessments:
Historical Context:
6.Evolution of AMS
Instrumentation Simplification:
Specialization for Carbon Analysis:
Commercial Availability:
Breakthroughs in Voltage Requirements:
Development of Carbon-Dedicated Systems:
Innovations in Sample Introduction:
Concerns and Future Directions:
7.Limitations of AMS
Sample Size Requirements:
Complex Sample Preparation:
Limited Automation:
Background Interference:
Sensitivity to Sample Composition:
Time-Consuming Analysis:
Limited Public Data:
8.Conclusion
9.Frequently Asked Questions (FAQs)
1. What is the primary use of AMS?
2. How accurate is AMS dating?
3. Why is AMS better than traditional radiocarbon dating?
4. What is the cost of AMS analysis?
5. Can AMS detect all isotopes?
Recent Posts
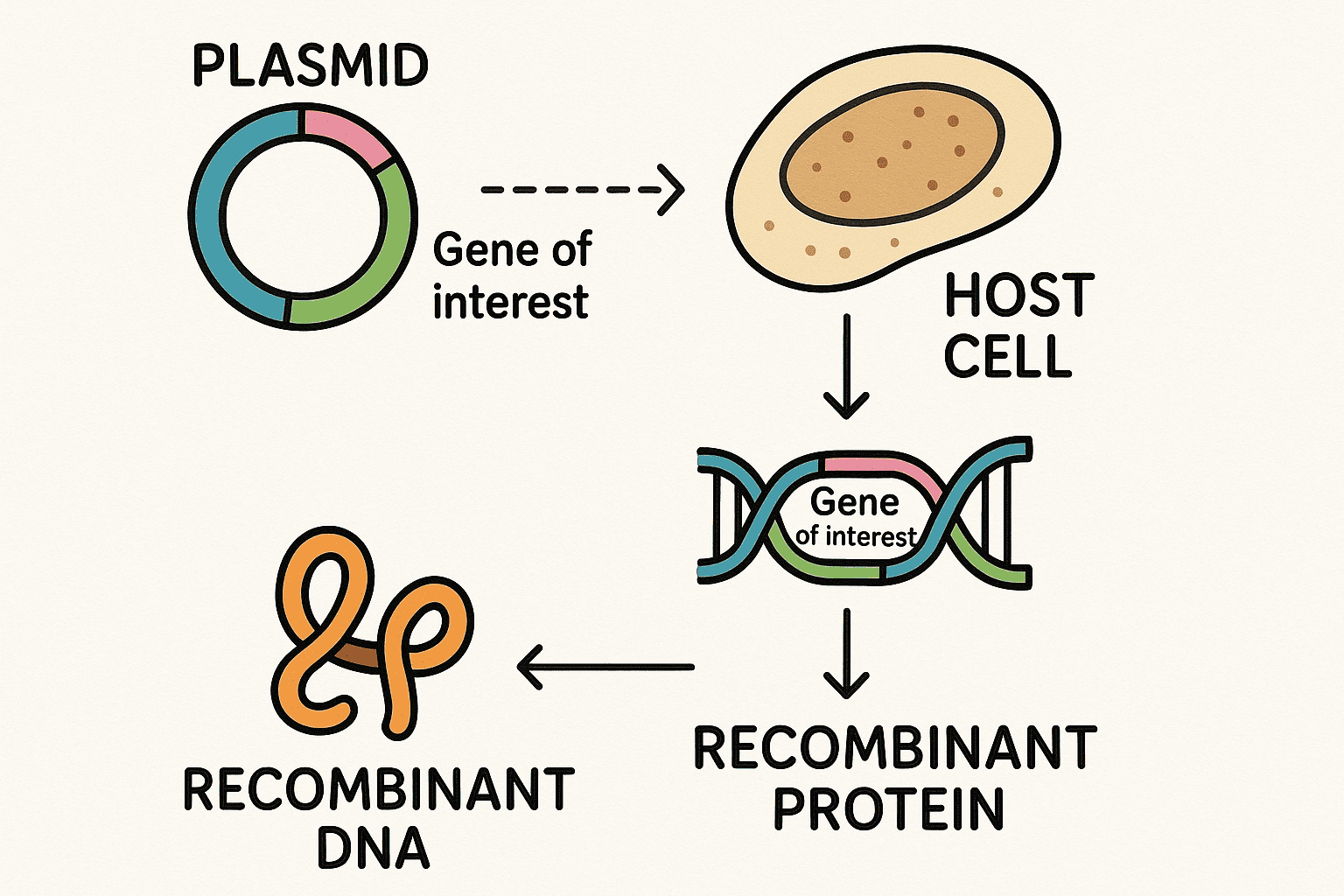
Mammalian expression systems enable the production of complex, functional recombinant proteins with proper folding and post-translational modifications. These systems are ideal for studying human proteins in a near-native environment, offering advantages in scalability, gene delivery, and purification. HEK293 and CHO cells remain the most widely used hosts, supporting both transient and stable expression strategies for academic and pharmaceutical applications.
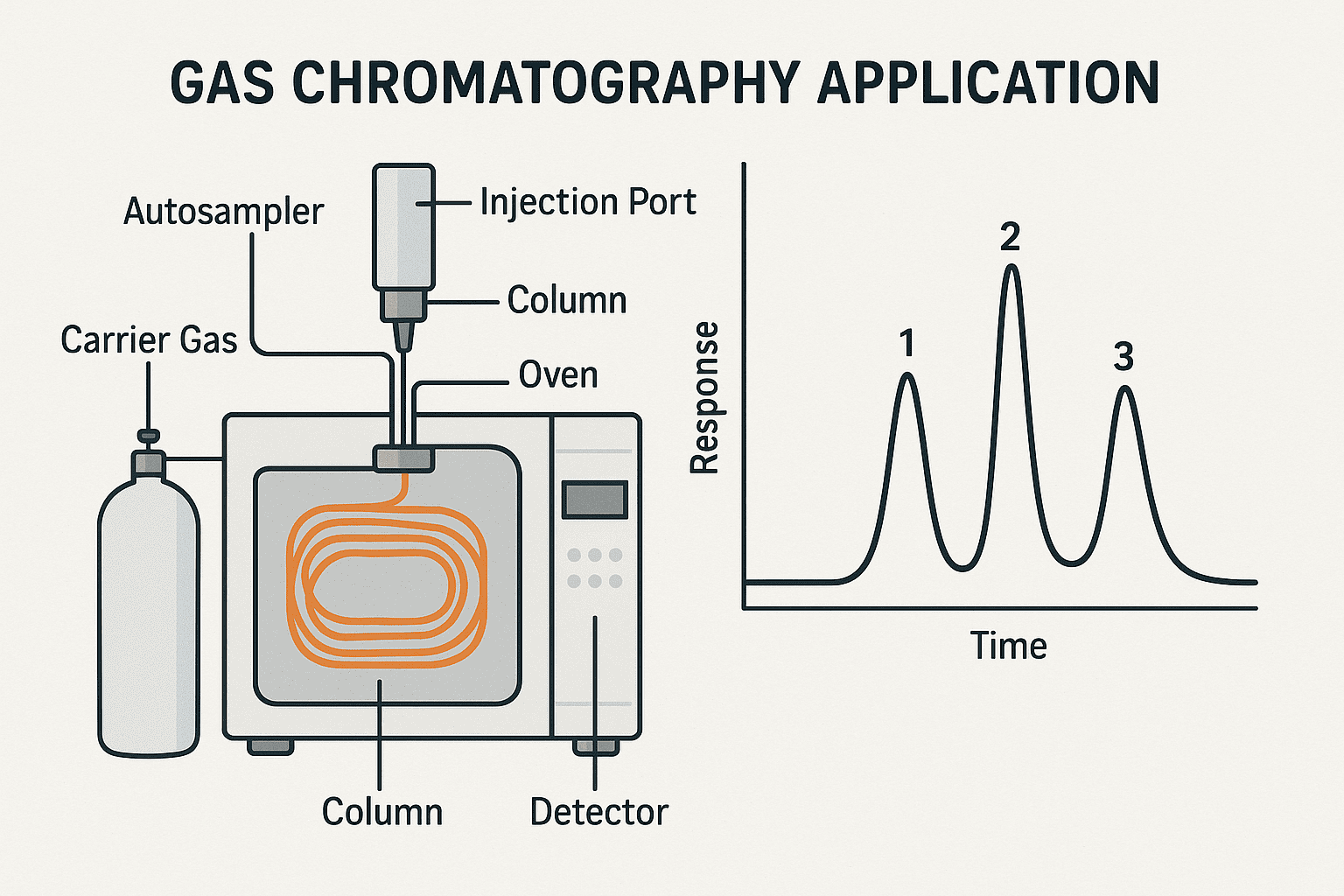
Gas Chromatography (GC) stands as one of the most powerful and versatile analytical techniques used to separate and analyze compounds in complex mixtures. At its core, GC enables the identification and quantification of chemical substances based on their molecular composition and retention behaviors during migration through a chromatographic column.
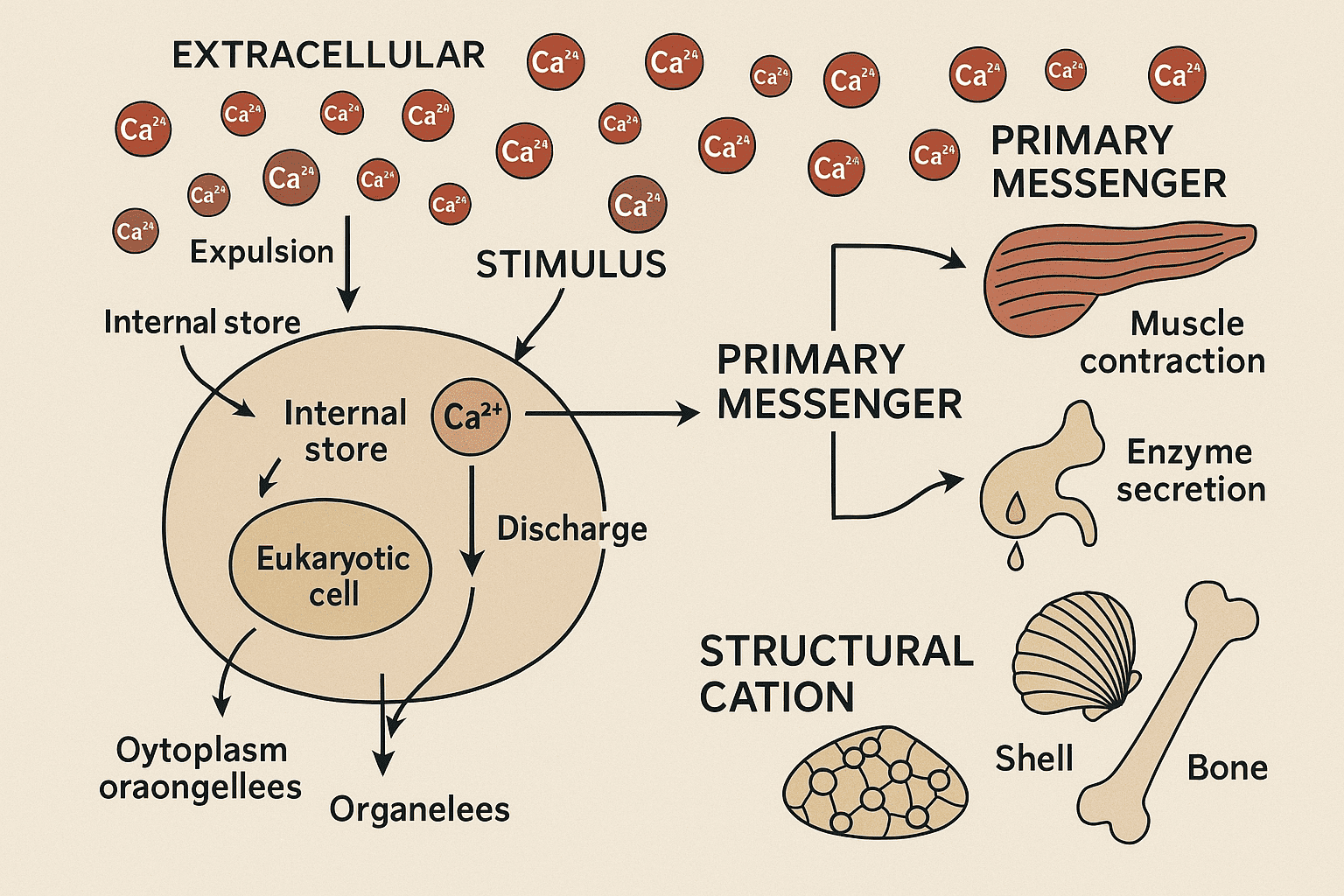
Calcium plays a crucial role as a specific cation, Ca2+, in cellular functions. It is expelled by all cell cytoplasm either into the extracellular environment or into internal reservoirs. From these reserves, it is discharged by stimuli from outside eukaryotic cells into the cytoplasm and organelles, where it triggers numerous processes.
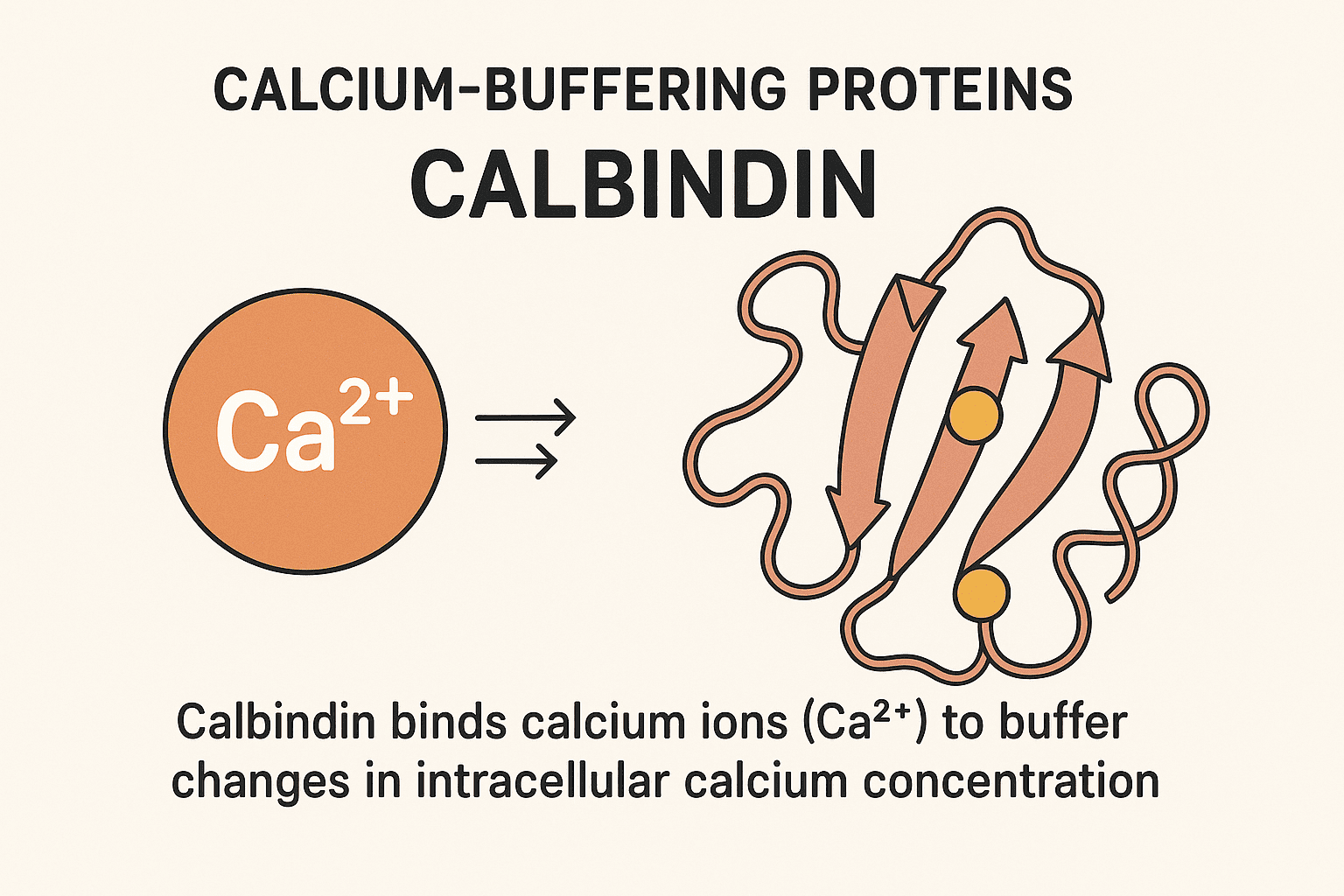
Calbindin-D28k and D9k are calcium-binding proteins that help regulate calcium levels and protect cells from damage. Though once seen as vitamin D-dependent, their expression is also influenced by other hormones and tissue-specific factors. While not essential for calcium absorption, they play important roles in calcium balance and cell health.
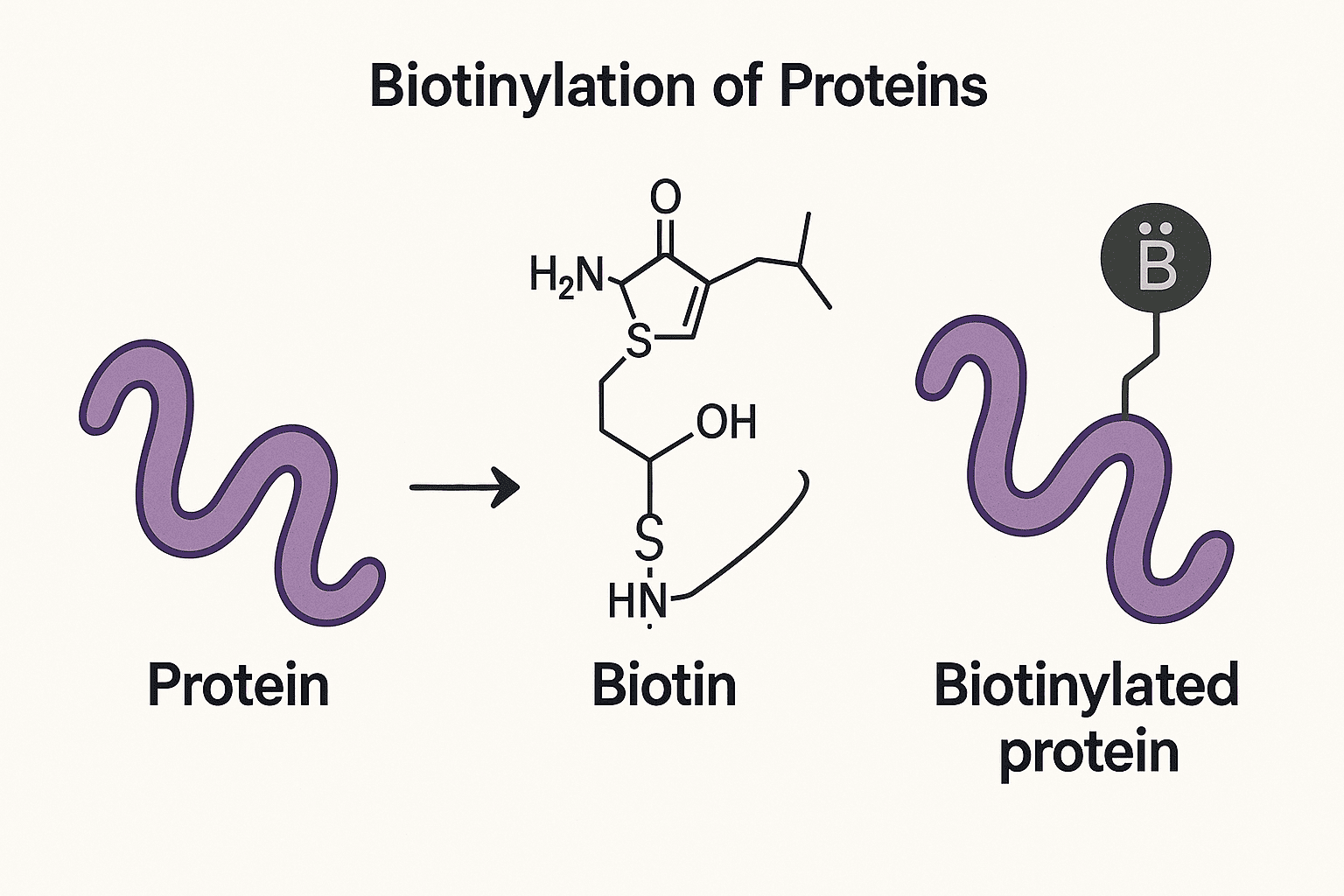
The biotinylation process of proteins involves the covalent attachment of biotin to an amino acid or carbohydrate component of the protein. This biotinylation specifically occurs in a category of proteins known as carboxylases. These enzymes play a crucial role in various metabolic pathways, such as amino acid metabolism, fatty acid production, and gluconeogenesis.